This article provides a comprehensive review of integrated photonic circuits (PIC) design methodologies for quantum computing applications, examining the historical context, material platforms, simulation tools, fabrication processes, and packaging techniques that underpin current approaches. Emphasizing challenges such as fabrication variability, phase stability, and loss reduction, it critically evaluates existing strategies and identifies gaps that must be addressed to enable scalable, reliable quantum photonic systems. The discussion extends to interdisciplinary skill requirements, policy frameworks, and international funding initiatives, underscoring the need for robust collaboration, standardized best practices, and continuous improvement in simulation models and design-for-manufacturing protocols. Concluding with a call to action, the article encourages targeted efforts in materials engineering, layout optimization, workforce development, and regulatory support to accelerate the transition of PIC-based quantum computing from visionary concepts to practical, high-fidelity technologies.
By: Javad Zarbakhsh, PhD, Cademix Institute of Technology, Austria
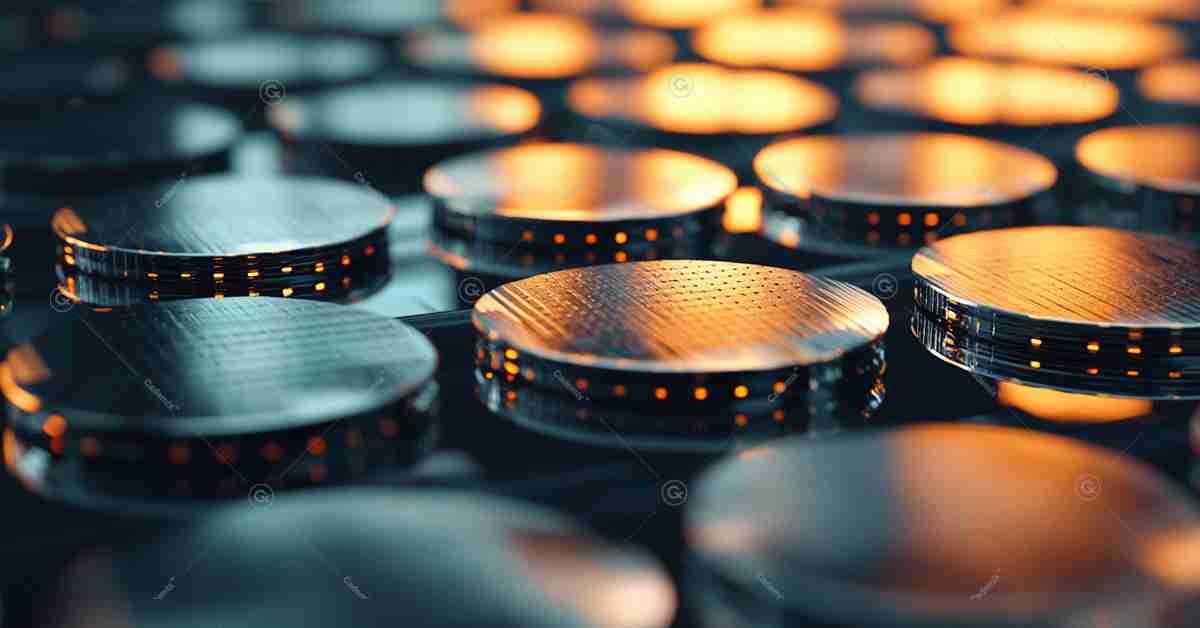
Table of Contents
Introduction
The pursuit of quantum computing applications has intensified over the past decade as researchers and engineers seek more efficient ways to leverage the fundamental principles of quantum mechanics to process information. Among the various hardware platforms being explored, integrated photonic circuits (PICs) have emerged as promising architectures, poised to meet the daunting requirements of scalability, coherence, and low operational overhead. These circuits, built upon semiconductor-based and other advanced photonic materials, allow photons to propagate and interact in highly controlled optical networks, thus enabling the encoding, manipulation, and readout of quantum information in stable photonic qubits. In contrast to conventional electronics, where electrons serve as information carriers, integrated photonics harnesses photons’ inherent immunity to thermal noise and their high bandwidth, making them suitable for low-loss, high-speed quantum operations.
Over the last decade, the field has progressed from simple waveguide couplers and beam splitters to increasingly complex photonic networks capable of implementing multi-qubit gates and interference-based protocols. Simultaneously, the advent of simulation tools, advanced fabrication techniques, and novel materials has supported the design of PICs with unprecedented complexity. While these advances are inspiring, the field faces significant challenges that demand critical attention. On the technological side, ensuring fabrication reproducibility, reducing propagation losses, and integrating active components such as detectors and modulators remain formidable tasks. From a design methodology standpoint, translating theoretical quantum protocols into fabricated PICs calls for sophisticated photonics simulation, robust layout optimization methods, and interdisciplinary expertise spanning physics, engineering, and material science. Equally essential are the organizational and cultural factors, from the skill gaps in the workforce to policy and funding frameworks, each influencing the trajectory and adoption of PIC-based quantum computing solutions.
In this comprehensive review, the aim is to dissect the design methodologies underlying integrated photonic circuits for quantum computing applications, while simultaneously casting a critical eye on the manifold challenges that lie ahead. The article explores the state of the art in PIC design, from conceptual frameworks and simulation approaches to material selection and fabrication processes, all viewed through the lens of quantum computing requirements. It provides a critical perspective rather than a purely celebratory account, highlighting where existing methodologies fall short and where further developments are needed. The discussion also extends beyond the technological sphere to consider how policy directives, EU-funded initiatives, training programs, and collaborative networks can steer the field toward robust, industrial-scale quantum photonic systems. In doing so, this review offers readers not only an understanding of the current methodologies but also a map of the critical challenges and opportunities that will define the next era of quantum computing through integrated photonics.
Historical Context and Conceptual Foundations of Integrated Photonic Circuits Design
The notion of integrated photonic circuits originated from the broader field of integrated optics, which took shape in the 1960s and 1970s when researchers sought to confine light in waveguides and manipulate it using on-chip components. Early integrated photonics lacked the sophistication we see today, but the foundational concepts of using planar dielectric structures to guide and control light provided a basis for the evolution of PIC design. Over time, advances in lithographic techniques and materials science enabled more complex optical devices, including arrayed waveguide gratings, ring resonators, and photonic crystal structures. These devices steadily found their way into telecommunications systems for wavelength division multiplexing and signal processing. By the early 2000s, with the advent of silicon photonics and CMOS-compatible fabrication techniques, integrated photonics became a mainstream technology for optical interconnects, biosensing, and other applications that benefitted from scaling optical functionalities onto a chip.
In quantum computing contexts, this historical trajectory has taken on new dimensions. Traditional integrated photonics aimed to route and process classical optical signals with minimal loss, but quantum photonics introduces stricter demands. The challenge is no longer just about guiding photons efficiently; it is about preserving quantum coherence and enabling precise quantum gates, entanglement generation, and state discrimination. In these quantum scenarios, the complexity of device design escalates, demanding not only intricate optical geometries but also materials and processes that support quantum-grade low loss, stable phase relationships, and the integration of single-photon detectors and emitters.
Conceptually, PIC design for quantum computing builds upon the foundational principle that linear optical networks combined with nonlinear elements, or suitable resource states, can implement quantum logic. This principle was famously articulated in the Knill-Laflamme-Milburn (KLM) scheme [https://doi.org/10.1038/35051009], which demonstrated how linear optics, projective measurements, and ancillary photons could achieve universal quantum computation. While KLM remains a theoretical touchstone, practical implementations have demanded new materials, improved engineering methods, and sophisticated simulation tools. Designers today rely on advanced electromagnetic solvers, optimization algorithms, and hybrid classical-quantum simulation approaches to map idealized quantum protocols onto chips. The conceptual foundation remains rooted in quantum optics and linear algebra, but its modern realization is intimately tied to the intricacies of nanofabrication, high-performance simulation software, and evolving quantum computing hardware paradigms.
Integrated Photonic Circuits and Quantum Computing Requirements
Quantum computing presents a unique set of demands on PIC design methodologies. Unlike classical optical interconnects that might tolerate minimal phase errors, quantum operations are exquisitely sensitive to loss, decoherence, and imperfections in device fabrication. A single photon acting as a qubit can encode quantum information in numerous degrees of freedom, including polarization, path, time-bin, and frequency. Preserving these encodings through the photonic chip is crucial, as any phase drift or mode mismatch can lead to computational errors. Additionally, scaling up from a handful of qubits to fault-tolerant architectures with hundreds or thousands of qubits is a core challenge. As quantum computing aims to solve problems beyond the capacity of classical supercomputers, it must rely on PICs that can be manufactured reproducibly, integrated with control electronics, and tested at scale.
Quantum computational protocols often hinge on implementing controlled interference patterns, nonlinear interactions mediated by measurement, or active phase modulation to enact logic gates. PIC designers must ensure that the chosen waveguide geometries, refractive index contrasts, and coupling regions perform reliably under these conditions. Low propagation loss is paramount: a few dB of excess loss scattered across multiple components can drastically reduce the fidelity of quantum operations. Similarly, phase stability is critical. In classical systems, small drift in refractive index or coupling coefficients can be tolerable, but in quantum circuits, such fluctuations can scramble fragile quantum states.
Another key requirement is compatibility with single-photon sources and detectors. Quantum computing protocols often demand on-chip single-photon sources based on quantum dots or parametric down-conversion, as well as superconducting nanowire single-photon detectors (SNSPDs) or avalanche photodiodes integrated directly onto the substrate. These requirements mean PIC design methodologies must consider thermal management, optical mode engineering for source and detector coupling, and the placement of these active elements in the circuit layout. Each consideration adds complexity and constrains the design space, forcing designers to engage with a diverse toolkit of simulation methods, lithographic techniques, and metrology methods.
State-of-the-Art PIC Design Methodologies
Modern PIC design methodologies have advanced beyond basic trial-and-error approaches. The integration of electromagnetic simulation tools, such as Lumerical [https://www.lumerical.com/] and Synopsys [https://www.synopsys.com/optical-solutions.html], as well as finite-difference time-domain (FDTD) solvers, beam propagation methods, and eigenmode expansion techniques, allows designers to model complex structures and predict device performance before fabrication. These tools help engineers optimize waveguide widths, bends, couplers, and resonators to minimize insertion loss and maximize mode overlap. They also aid in the placement of active components, helping to ensure that mode profiles match the operational wavelengths and polarization states of the quantum signals.
A central feature of state-of-the-art PIC design is the iterative feedback loop between simulation and experimental characterization. Designers can simulate an initial layout, fabricate a test chip through a foundry, measure its optical performance, then refine simulation parameters. This loop is particularly critical in quantum computing, where achieving desired unitary transformations depends on precise device parameters. The synergy between simulation and experimental feedback forms the backbone of modern design methodology, fostering continuous improvement and reliability.
To address the complexity of quantum circuits, some methodologies incorporate machine learning and inverse design. Algorithms can start from desired quantum unitary transformations and work backward to identify device geometries that implement these operations. This approach is particularly helpful in optimizing complex couplers and interferometers that would be challenging to design with conventional intuition. While still in its infancy, inverse design is already showing promise in delivering compact, high-performance components. However, such methods still face challenges in ensuring fabrication tolerance and aligning with available materials and foundry processes.
Material Platforms and Silicon Photonics
The choice of material platform sets the stage for the entire PIC design process. Silicon photonics has emerged as a leading candidate due to its compatibility with CMOS fabrication and the existing infrastructure for large-scale manufacturing. Silicon photonics platforms offer high refractive index contrast and allow dense integration of waveguides and components. Yet silicon’s indirect bandgap precludes it from serving as an efficient light source, necessitating hybrid approaches that incorporate III-V semiconductors for gain elements or rely on external laser coupling. Despite this shortcoming, silicon photonics remains attractive for quantum computing due to its maturity, scalability, and the possibility of leveraging advanced microelectronics packaging processes.
Beyond silicon, other materials such as silicon nitride, lithium niobate, and indium phosphide present alternative advantages. Silicon nitride waveguides, for example, exhibit lower propagation loss than silicon, making them suitable for quantum states that must travel across many components. Lithium niobate brings strong electro-optic modulation capabilities, potentially enabling on-chip control of quantum states, while indium phosphide supports integrated gain elements, which might facilitate the generation and manipulation of single photons on chip. Each platform involves trade-offs in terms of loss, integration complexity, and component availability. The choice of material often depends on the specific quantum computing protocol and the desired level of integration and complexity.
As researchers push toward practical quantum computing applications, hybrid integration techniques are gaining traction. These techniques combine different materials and device functionalities on the same substrate, utilizing adhesive bonding or heterogeneous integration to integrate, for instance, a silicon photonics platform with a quantum dot source grown in a III-V material system. This approach can yield highly functional chips, but also increases design complexity, as each material addition may introduce new losses, alignment challenges, and reliability concerns. Considering these complexities early in the design process is crucial, and simulation tools must incorporate material-specific models to predict device behavior accurately.
Fabrication Processes and the Challenges of Scalability
Fabrication stands at the heart of integrated photonic circuit design. Even the most elegant simulated designs mean little if they cannot be fabricated reproducibly and cost-effectively at scale. Traditional photonic device fabrication involves a sequence of lithographic steps, etching, deposition of dielectric layers, metallization for electrodes, and packaging. While these processes have become routine in research cleanrooms and commercial foundries, quantum computing PICs demand stricter tolerances and reproducibility.
One persistent challenge is minimizing sidewall roughness in waveguides, as scattering from rough interfaces leads to propagation losses and dephasing. Achieving smooth interfaces often requires optimized lithography techniques and sometimes post-processing steps like thermal annealing or chemical polishing. Another challenge is ensuring uniformity across the wafer. As circuits scale to larger chip areas and incorporate more devices, slight variations in etch depth or layer thickness can cause performance discrepancies. These variations translate to non-uniform phase shifts, coupling ratios, and losses, hampering the faithful implementation of quantum gates across multiple qubits.
To address these challenges, designers must work hand in hand with fabrication engineers, iterating designs to account for expected fabrication tolerances. Design-for-manufacturing strategies, well-known in microelectronics, are increasingly applied to photonics. Such strategies might involve deliberately widening waveguides, using standardized building blocks, or including test structures that provide post-fabrication feedback. Despite these measures, the complexity and sensitivity of quantum PICs raise the question of whether today’s fabrication methodologies are sufficient. If not, new fabrication technologies or techniques will need to emerge, such as improved electron-beam lithography resolution, nanoimprint lithography, or self-assembled material structures that automatically yield low-loss optical channels.
Packaging and Coupling Considerations
Designing a PIC is only half the battle; coupling light in and out of the chip, packaging the device, and ensuring it operates reliably under real-world conditions represent equally formidable tasks. In quantum computing applications, packaging challenges extend beyond robust fiber-to-chip coupling. Designers must integrate single-photon detectors, control electronics, and thermal stabilization systems, all within a compact and stable package that can operate at cryogenic temperatures if necessary. For instance, superconducting single-photon detectors require cryogenic operation, which complicates the packaging approach, as the materials and adhesives used must maintain their integrity and alignment at low temperatures.
Coupling quantum photonic chips to optical fibers or waveguides that deliver single photons from external sources is also challenging. Imperfect coupling reduces the overall efficiency and can introduce mode mismatches or reflections, degrading quantum fidelity. Although various techniques exist, such as edge coupling, grating couplers, or tapered waveguides, none are perfect. Each technique involves balancing factors like fabrication complexity, wavelength sensitivity, polarization dependence, and insertion loss. Packaging solutions often look toward established silicon photonics solutions, but the added complexity of quantum signals and the need for near-lossless coupling pushes the envelope further.
A promising approach involves photonic packaging with 3D-printed micro-optical components or laser-written waveguide circuits that transition between fiber modes and on-chip modes seamlessly. While these techniques show promise, they must be perfected to handle quantum states. Design methodologies must thus consider packaging constraints from the outset, ensuring that waveguide modes, coupling interfaces, and device positions align with known packaging solutions. This integration of design and packaging considerations reduces the risk of discovering insurmountable coupling losses only after the chip is fabricated.
Reliability, Testing, and Long-Term Stability
Reliability plays a crucial role in PIC-based quantum computing systems, which must maintain their operational parameters over extended periods. While classical optical networks can tolerate minor fluctuations, quantum computing demands long-term phase stability, low drift, and consistent performance. Testing a PIC designed for quantum applications involves more than measuring insertion loss or bandwidth. It may require characterizing single-photon arrival times, heralding efficiencies, two-photon interference visibilities, and other quantum metrics that go beyond standard telecom testing protocols.
Ensuring reliability involves both pre- and post-fabrication strategies. On the pre-fabrication side, designers must incorporate margins into their simulations and rely on robust design rules that account for fabrication variability, temperature fluctuations, and material aging. On the post-fabrication side, active stabilization mechanisms, such as thermo-optic or electro-optic tuning elements, can compensate for drift. However, these tuning elements add complexity and overhead to the design, underscoring the importance of initial design choices that minimize the need for corrections.
From a testing perspective, measuring quantum performance metrics typically requires sophisticated experimental setups and low-noise detectors. As PICs become more integrated and complex, on-chip diagnostic structures and calibration elements may help streamline testing procedures. Designers might include reference waveguides, interferometers, or beam splitters whose performance can be monitored to assess the overall stability of the device. Over time, these feedback mechanisms can guide incremental improvements and reduce the reliance on external laboratory infrastructure for testing. Nevertheless, the field still lacks standardized testing protocols for quantum PICs. Without such protocols, comparing results from different groups or different foundries becomes difficult, hindering the collective progress of the field.
Organizational, Cultural, and Skill-Related Challenges
The complexity of PIC design for quantum computing extends beyond the technological domain into organizational and cultural spheres. Historically, photonics research groups have been spread across academia, industry, and government labs, each with its own design methodologies, fabrication facilities, and performance metrics. Integrating these groups to achieve faster progress and effective knowledge transfer is no small feat. Organizational challenges include forging productive collaborations between material scientists, quantum theorists, device engineers, and foundry technicians. Cultural differences between academic and industrial stakeholders can also slow the translation of laboratory breakthroughs into commercially viable technologies.
On the skill side, designers face a broad knowledge requirement. Successful PIC engineers must understand quantum optics, solid-state physics, simulation software, semiconductor fabrication, and packaging techniques. They must also be adept at project management and communicate effectively with professionals from other domains. Bridging these skill gaps often requires continuing education programs, workshops, and interdisciplinary courses. Universities and research institutions have begun to establish specialized training modules, online courses, and internship programs with quantum photonics start-ups or established photonic foundries. Still, the pace at which the field is evolving means skill requirements are continuously shifting, placing pressure on both individuals and institutions to keep curricula and training programs up to date.
Policy, Funding, and the Role of International Initiatives
Policy frameworks and funding mechanisms play a significant role in shaping PIC design methodologies for quantum computing. Large-scale funding initiatives in the European Union, United States, and Asia have identified integrated photonics and quantum technologies as strategic priorities. EU-funded projects often aim to align research efforts across universities, SMEs, and large companies, facilitating technology transfer and scaling. Funding calls encourage not only technological innovation but also workforce development, standardization efforts, and the creation of shared infrastructure, such as open-access foundries [https://opencircuitdesign.com/], testing facilities, and design libraries.
However, policy measures sometimes lag behind the rapid advances in technology, and regulations or standards that would streamline PIC design for quantum computing may not yet exist. The field could benefit from standardized design kits, material libraries, and open-source simulation tools that lower entry barriers for new researchers and companies. Funding agencies can incentivize such community-driven efforts by emphasizing reproducibility, transparency, and knowledge sharing in their evaluation criteria. Collaborative networks, such as European COST actions and Quantum Flagship initiatives [https://qt.eu/], are stepping in to connect disparate players, but more systematic frameworks are needed.
These policy and funding dimensions are not mere formalities. They directly impact the efficiency and direction of research and development. Without coordinated policies, critical challenges in fabrication, packaging, and reliability may not receive adequate attention. Similarly, without proper funding and incentives, small companies or start-ups developing niche technologies may struggle to survive, even if their innovations are essential to the larger ecosystem. Ensuring that funding aligns with long-term goals and that policy frameworks are agile enough to evolve with the technology is crucial for fostering sustained progress.
Towards Improved Methodologies: Prospects and Critical Paths
The road to robust and scalable PIC-based quantum computing solutions is paved with both opportunities and unresolved challenges. On the technological front, improvements in fabrication techniques, such as better lithographic resolution, advanced etch chemistries, and self-assembled photonic structures, could dramatically reduce losses and increase uniformity. Similarly, breakthroughs in material science may yield platforms that support low-loss transmission, integrated gain, and strong nonlinearities, opening new pathways for quantum photonic circuits.
On the simulation and design side, better integration of inverse design algorithms, machine learning, and multi-physics simulation approaches could drastically improve device performance and design speed. By feeding back experimental data into these algorithms, designs can evolve rapidly, converging on solutions that are inherently more tolerant to fabrication variability. The adoption of standardized building blocks, coherent software-tool ecosystems, and shared design libraries would also streamline the process.
Yet progress in these directions will require collaborative efforts, continued funding, and a willingness to confront the systemic barriers that currently impede rapid development. The involvement of standardization bodies, the publication of comprehensive design kits, and an emphasis on reproducibility will help build trust and accelerate adoption. Organizationally, interdisciplinary consortia and collaborative partnerships between academia, industry, and government labs must become the norm rather than the exception. Each partner can bring unique strengths—fundamental research expertise, manufacturing capabilities, or market intelligence—that collectively propel the field forward.
Conclusion and Call to Action
Integrated photonic circuits hold tremendous promise as the foundational hardware for quantum computing applications. They combine the stability of photons as information carriers with the scalability and integration potentials inherited from the semiconductor industry. Yet the path to a fully integrated, large-scale quantum computing system remains fraught with challenges. Design methodologies must evolve to incorporate not only electromagnetic simulations but also considerations of material properties, fabrication tolerances, packaging constraints, and quantum-specific performance metrics. PIC designers must manage these complexities while remaining agile enough to incorporate new techniques, materials, and concepts as the field progresses.
This comprehensive review reveals that no single breakthrough will solve the multifaceted challenges of PIC design for quantum computing. Instead, collective efforts are needed. Researchers, engineers, and policymakers must work together, guided by robust simulation methods, design-for-manufacturing strategies, and incentive structures that support skill development and standardized best practices. Funding agencies and policy frameworks must encourage collaborative networks, open-access foundries, and training programs that build a workforce fluent in both photonics and quantum principles.
The call to action is clear: stakeholders across the spectrum must invest in refining PIC design methodologies. This includes developing better simulation tools and model libraries, improving fabrication processes for lower loss and higher reproducibility, creating packaging solutions that integrate seamlessly with quantum devices, and establishing testing protocols that measure quantum-specific performance metrics. Education and skill-building initiatives will ensure that the talent pool keeps pace with the technology. International collaborations and well-structured policy measures will ensure that the technological advances not only persist in the laboratory but also translate into valuable solutions. By addressing these challenges methodically and coherently, the integrated photonics community can play a pivotal role in bringing quantum computing from a visionary concept to a tangible and transformative technology.
References and Further Reading
Knill, E., Laflamme, R., & Milburn, G. J. (2001). A scheme for efficient quantum computation with linear optics. Nature, 409(6816), 46–52. [https://doi.org/10.1038/35051009]
Lumerical Inc. Lumerical Photonic Design Tools. [https://www.lumerical.com/]
Synopsys Inc. Photonic Solutions. [https://www.synopsys.com/optical-solutions.html]
European Quantum Flagship. [https://qt.eu/]
Open Circuit Design. [https://opencircuitdesign.com/]
Bogaerts, W., et al. (2014). Silicon photonics circuit design: methods and tools. Laser & Photonics Reviews, 8(1), 139–158. [https://doi.org/10.1002/lpor.201300084]
Politi, A., Matthews, J. C., Thompson, M. G., & O’Brien, J. L. (2009). Integrated quantum photonics. IEEE Journal of Selected Topics in Quantum Electronics, 15(6), 1673–1684. [https://doi.org/10.1109/JSTQE.2009.2026060]
Perez, D., Gasulla, I., & Capmany, J. (2017). Field-programmable photonic arrays. Optics Express, 25(3), 282–294. [https://doi.org/10.1364/OE.25.000282]
Soref, R. (2010). The past, present, and future of silicon photonics. IEEE Journal of Selected Topics in Quantum Electronics, 16(1), 167–176. [https://doi.org/10.1109/JSTQE.2009.2035196]