This article critically examines the challenges and opportunities associated with waveguide integration in next-generation quantum photonic devices. It explores the stringent requirements imposed by quantum coherence, discusses the complexities introduced by material platforms, PIC fabrication, and optical packaging, and evaluates the feasibility of emerging integration techniques. Emphasizing the importance of fabrication tolerances, organizational skill gaps, and policy frameworks, the analysis reveals a landscape where technological innovation, interdisciplinary collaboration, and standardized practices converge to enable scalable, high-fidelity quantum photonic systems. The review ultimately encourages continued research, education, and policy alignment to address persistent barriers and guide waveguide integration from conceptual design to stable, manufacturable quantum photonic architectures.
By: Dr. Javad Zarbakhsh, Cademix Institute of Technology, Austria
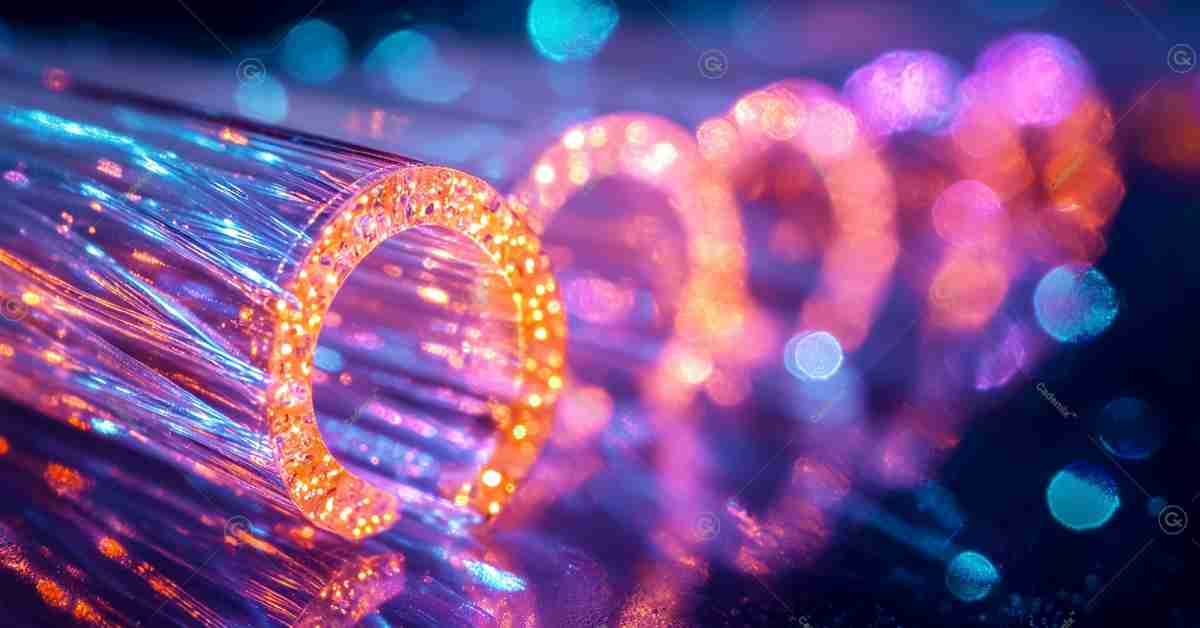
Table of Contents
Introduction
The integration of waveguides into next-generation quantum photonic devices stands as one of the more intricate and consequential challenges facing the field of integrated photonics. Although waveguide integration might appear to be a relatively narrow technical pursuit, the complexity and ramifications of this process extend far beyond the mere guiding of light through confined optical channels. Instead, it intersects with virtually every stage of quantum photonic device development, from the conceptual design of quantum optical circuits, through the intricacies of photonic integrated circuit (PIC) fabrication, to the final steps of optical packaging and long-term reliability testing. These quantum photonic devices, which aim to exploit the principles of quantum mechanics to process information, sense signals, or enable secure communication, represent a technological frontier where performance is not merely incremental but defined by thresholds of coherence, fidelity, and scalability. Waveguide integration lies at the heart of these thresholds, dictating in large measure whether quantum photonic systems can transition from laboratory prototypes to robust, manufacturable technologies.
In classical optical communication systems, waveguides can be engineered with relative ease to transmit signals over long distances or route optical pulses across telecommunications networks. The constraints in quantum photonic devices, however, are far more stringent. Quantum states encoded in photons are delicately balanced superpositions, and even minuscule losses, phase shifts, or coupling inefficiencies introduced by imperfect waveguides can degrade quantum fidelity. The challenge intensifies when scaling from a few components to large, multi-element PICs designed for complex quantum algorithms. Moreover, waveguide integration must consider not only the guiding layers and interfaces but also how these optical conduits align with quantum emitters, single-photon detectors, and other photonic components that collectively form the backbone of the device. The entire ecosystem becomes a delicate interplay of materials, geometries, refractive index contrasts, fabrication methods, and operational environments.
Yet this challenge is not without its opportunities. Researchers have made notable strides in developing specialized materials, improving lithographic precision, and implementing advanced simulation methods to refine waveguide designs. Emerging techniques in quantum photonic integration, inspired by inverse design algorithms and machine learning, promise to push performance further. Interdisciplinary collaborations, supported by international funding and guided by policy frameworks, are directing efforts to address organizational and cultural barriers that slow the translation of academic research into industrial-scale products. Alongside these technological initiatives, there is a growing recognition of the need for skill development and training, ensuring that the workforce can navigate the complexities of PIC fabrication and optical packaging while understanding the quantum optical principles that make these devices unique. This review sets out to examine the multifaceted challenges in waveguide integration, consider the potential solutions, and critically evaluate the feasibility and scalability of emerging integration techniques. The emphasis lies on understanding the barriers and identifying paths forward, rather than presenting definitive solutions, given the rapidly evolving state of this field.
Historical Evolution of Waveguide Integration in Photonics
Waveguide integration is not a new concept. The field of integrated optics, dating back to the 1960s and 1970s, introduced planar waveguides as a way to confine and manipulate light within substrates such as silica on silicon. Early integrated photonics platforms focused largely on classical applications, including optical communications and signal processing. In these early days, waveguides were relatively thick structures, and the alignment tolerances and losses, while critical, did not approach the exacting standards required for quantum photonic devices. The emphasis was on reducing insertion loss, improving wafer-scale uniformity, and increasing component density, but not necessarily on preserving quantum coherence or supporting single-photon-level operations.
Over time, the introduction of silicon photonics and other advanced platforms brought waveguide integration to the forefront of modern PIC technology. By exploiting CMOS-compatible fabrication methods, engineers could realize complex circuits that integrated waveguides with modulators, filters, detectors, and even lasers. The objective at that stage often revolved around improving speed, reducing footprint, and achieving cost savings through volume production. As the field matured, researchers demonstrated wavelength-division multiplexing systems, photonic switches, and reconfigurable optical circuits. These achievements laid an important foundation, as they clarified what is possible when waveguide integration meets mature semiconductor processes.
It is against this historical backdrop that quantum photonics has emerged. Quantum computing, quantum communication, and quantum sensing demand unprecedented performance levels from integrated waveguides. The generation and manipulation of single photons, the implementation of linear optical gates, and the creation of entangled states rely on waveguides that introduce minimal photon loss, exhibit stable phase relations, and couple efficiently to ancillary components. The quantum domain thus inherits the lessons learned in classical integrated optics but applies them to a regime where even a fraction of a dB of additional loss or a small phase error can compromise the entire quantum operation. The historical evolution shows that waveguide integration, while once a challenge of engineering precision, is now a delicate dance of quantum design principles, nanoscale fabrication, and interdisciplinary coordination.
Defining the Requirements for Quantum Photonic Devices
Before delving deeper into the challenges, it is worth considering what quantum photonic devices demand from waveguide integration. Quantum devices are built on the delicate manipulation of quantum states, and photons serve as a robust carrier of quantum information due to their low decoherence and ease of traveling across optical networks. However, the quantum advantage is only realized if the photon’s quantum state remains well-defined and coherent through the entire processing sequence. In practical terms, this requirement translates to stringent constraints on loss, dispersion, coupling efficiency, and mode purity within waveguides.
For devices that rely on path-encoded qubits or interferometric operations, waveguide propagation losses must be minimized to maintain high fidelity. Even a small fraction of loss over multiple components can quickly reduce success probabilities and gate fidelities, making complex quantum circuits impractical. Similarly, the refractive index contrast and waveguide geometry must be carefully chosen to ensure that the mode profiles are stable and reproducible. In quantum devices, small phase variations can alter interference patterns, thus changing the outcome of quantum logic operations. This sensitivity means that thermal fluctuations, fabrication-induced roughness, and even subtle stress in the substrate can degrade performance. Integrating waveguides that operate at cryogenic temperatures or require integration with superconducting nanowire single-photon detectors adds another layer of complexity, as materials must remain stable and compatible in low-temperature regimes.
One cannot consider waveguide integration for quantum photonic devices without acknowledging that these circuits often require integration with quantum emitters and single-photon sources. Such emitters may be embedded in materials like diamond (with nitrogen-vacancy centers) or semiconductor quantum dots. The waveguide design must ensure that the quantum emitters couple efficiently into the guiding mode, that the emission wavelength and polarization are matched, and that any scattering or mode conversion does not degrade the quantum state. This level of integration goes beyond waveguide design in isolation, weaving it into a broader tapestry of PIC fabrication and optical packaging challenges.
Material Platforms and PIC Fabrication Complexities
Choosing the right material platform for waveguide integration in quantum photonic devices presents both an opportunity and a hurdle. Silicon photonics, for instance, has garnered significant attention due to its compatibility with existing semiconductor fabrication infrastructure and the possibility of large-scale integration. Silicon-on-insulator waveguides boast high index contrast, which can facilitate compact device footprints. Yet high index contrast can also exacerbate scattering losses from sidewall roughness and make mode coupling more challenging. Additionally, silicon’s indirect bandgap means it cannot serve as a light source, so external lasers or hybrid integration with III-V materials must be employed, increasing complexity.
Silicon nitride waveguides have emerged as an attractive alternative for certain quantum photonic applications because they often yield lower propagation losses. This advantage can be crucial when dealing with single-photon signals and delicate quantum states. Nevertheless, these platforms might present difficulties in achieving certain functionalities or integrating active components. Similarly, lithium niobate offers excellent electro-optic properties, which can be beneficial for on-chip quantum control, but integrating lithium niobate waveguides into a larger PIC with detectors and other functionalities is not always straightforward. Indium phosphide provides access to on-chip gain media and quantum dot emitters, potentially unlocking integrated single-photon sources, but fabrication complexity and cost can be higher, and material compatibility must be carefully managed.
In quantum photonic devices, hybrid integration—the process of combining different material platforms on a single chip—is becoming an increasingly common strategy. By bonding a thin film of a III-V semiconductor on a silicon substrate, it is possible to integrate single-photon sources directly onto a waveguide platform. Similarly, incorporating nonlinear crystals or other specialty materials can enable on-chip entangled photon generation or wavelength conversion. While hybrid integration opens up unprecedented functionalities, it also multiplies the challenges. Each interface must be carefully managed to minimize reflection, scattering, and mode mismatch, and each added material brings its own thermal expansion coefficients, propagation characteristics, and lithographic sensitivities. The complexity of PIC fabrication thus grows exponentially, and waveguide integration must be thought of not as a single step but as an iterative process that interacts with every other fabrication stage.
The Role of Simulation and Design Methodologies
Advanced simulation and design methodologies have made it possible to model waveguide structures and predict their performance before fabrication. Tools like finite-difference time-domain solvers, eigenmode expansion methods, and beam propagation simulations allow engineers to optimize waveguide geometries to reduce losses, manage dispersion, and match mode profiles to desired functionalities. For quantum applications, simulations often go beyond classical electromagnetic field distributions, incorporating quantum mechanical considerations or running classical simulations under conditions that approximate single-photon behavior.
However, no simulation approach is perfect, and one of the core challenges lies in bridging the gap between idealized numerical models and real fabricated devices. Simulations rely on assumed refractive indices, layer thicknesses, and smooth boundary conditions, whereas actual fabrication inevitably introduces deviations. Moreover, the complexity of quantum photonic devices, with their multi-layer stacks, embedded emitters, and heterogeneous integration, means that simulating the entire system at once is computationally demanding. The result is that designers often rely on simplified models or break down the device into subproblems, each simulated separately. This segmentation can miss coupling effects between different parts of the circuit.
In recent years, inverse design techniques have gained traction. Rather than starting with a preconceived geometry and tuning parameters, inverse design algorithms search through a large design space to find waveguide structures that yield the desired optical response. While this approach has generated devices with improved performance and compact footprints, it has not yet fully addressed the quantum-specific challenges. Inverse design solutions might be highly sensitive to fabrication imperfections, or they might yield geometries that are difficult to realize with current lithographic processes. Thus, while advanced simulation and design offer pathways to better waveguide integration, they also highlight the need for closer interplay between theoretical design and experimental fabrication.
Fabrication Tolerances and Yield Issues
Fabrication tolerances represent a recurring theme in the effort to integrate waveguides for quantum photonic devices. High-fidelity quantum operations often require that multiple waveguides and components behave identically or follow tight specifications. A small variation in waveguide width or etch depth across the wafer can introduce phase errors or loss differences that accumulate as photons travel through complex circuits. In mass production, yield becomes a paramount concern. If a large fraction of PICs fail to meet strict quantum performance criteria due to fabrication-induced variations, the technology will struggle to scale and be economically viable.
One approach to addressing fabrication tolerances is to incorporate tuning elements, such as thermo-optic or electro-optic modulators, that can compensate for small variations post-fabrication. However, tuning introduces additional complexity, power consumption, and potential instability. Another strategy is to define robust design rules that ensure some degree of fabrication insensitivity. For example, choosing waveguide geometries that are less sensitive to sidewall roughness or temperature changes can help. Still, this often comes at the cost of increased footprint or reduced flexibility.
In some cases, foundries have begun to offer standardized platforms with well-characterized waveguide libraries, similar to the process design kits used in microelectronics. These standardizations can reduce variability and improve reproducibility, allowing designers to rely on tested components rather than starting from scratch. Yet the quantum domain, with its specialized needs, might require more stringent standardizations or even dedicated quantum photonic foundries. The organizational challenges in creating such specialized infrastructures, along with cultural differences in how academic, industrial, and government labs operate, continue to slow this process.
Optical Packaging: From Chip to System
Waveguide integration does not end at the chip level. Optical packaging, which involves coupling light from external fibers or free-space optics into the waveguides on the chip, aligning different chips together, and integrating detectors and control electronics, represents another layer of complexity. Packaging can introduce additional insertion loss, alignment challenges, and reliability concerns. For quantum photonic devices, packaging must preserve the quantum state and maintain phase stability over time. Achieving stable packaging at cryogenic temperatures, when required by superconducting detectors, is even more difficult since materials respond differently at low temperatures, and mechanical stresses can build up.
In some cases, three-dimensional integration approaches are being explored, where waveguides are stacked in multiple layers or integrated with 3D-printed micro-optics to facilitate coupling. Such approaches can reduce the total footprint and improve coupling efficiencies but also pose new challenges in materials compatibility and design complexity. Another potential solution is the use of photonic wire bonds, a technique in which waveguides are “written” in three dimensions using laser-based methods, connecting different chips without the need for traditional packaging interfaces. While these technologies are promising, they are still maturing, and their suitability for quantum photonic devices remains to be thoroughly validated.
The coupling of quantum photonic chips to external quantum systems, such as trapped ions, neutral atoms, or solid-state qubits, adds another dimension to packaging challenges. Waveguides must be designed to efficiently route photons to and from these external platforms, often with stringent polarization and spectral requirements. Such coupling often involves matching the mode profiles and spatial arrangements of external quantum systems, and even slight deviations in waveguide geometry or position can degrade coupling efficiencies. These considerations highlight that waveguide integration must be thought of not just as a chip-level problem, but as part of a larger, system-level challenge.
Reliability and Long-Term Performance
While much effort is placed on achieving optimal waveguide integration during initial fabrication and packaging, the long-term reliability of these devices is equally important. Quantum computing or communication systems may need to operate for extended periods without recalibration. Small environmental fluctuations, such as temperature changes, mechanical vibrations, or radiation-induced defects, can alter waveguide properties over time. If performance drifts, the integrity of quantum operations can degrade, leading to errors and reduced system reliability.
Ensuring reliability involves both the material choice and the device architecture. Some materials are more stable than others, and certain geometries are less sensitive to external perturbations. Protective coatings, hermetic packaging, and on-chip monitoring elements can help maintain stable conditions. For example, a reference interferometer integrated on the same chip can provide a signal that indicates phase changes over time, allowing for active compensation. Such solutions require careful forethought and often add extra complexity and cost.
Another subtle factor is the evolution of defects within the waveguides. Over time, tiny cracks, color centers, or absorption centers might form due to exposure to high-intensity light or environmental factors. These defects can increase propagation losses or create scattering centers that degrade quantum coherence. Understanding and mitigating defect formation will require insights from materials science, surface chemistry, and even radiation physics. As quantum photonic devices move from laboratory prototypes into commercial products, reliability and long-term stability will likely rise to the top of the priority list.
Organizational, Cultural, and Skill Dimensions
Beyond the technical challenges, waveguide integration in quantum photonic devices is influenced by organizational and cultural factors. The interdisciplinary nature of the field means that teams often include quantum physicists, photonic engineers, material scientists, and industrial experts in fabrication and packaging. Each of these stakeholders brings unique priorities and expertise. Effective collaboration requires a shared language, aligned goals, and transparent communication channels. However, institutional structures may not always support such interdisciplinary efforts. Academic incentives often reward fundamental research over applied engineering, while industry players prioritize manufacturability and profitability.
Cultural differences can also slow down the adoption of best practices. A researcher trained in a quantum optics lab might be unfamiliar with the stringent design rules and documentation standards required by a commercial foundry. Conversely, a fabrication engineer might find quantum operational requirements vague or overly idealized. Bridging these divides often involves training programs, internships, and workshops that expose individuals to complementary skill sets. Over time, the field will need professionals who are comfortable working at the intersection of quantum theory, optical design, and semiconductor fabrication.
Skill development initiatives can include specialized university courses in quantum photonics, collaborative PhD programs with industry partners, or government-funded training projects. These efforts ensure that the workforce remains adaptable and can implement emerging techniques in waveguide integration. Without addressing these human and organizational issues, even the most advanced technologies will struggle to find their way to market.
Policy, Funding, and Standardization Initiatives
Policy measures and funding frameworks play a significant role in shaping the landscape of waveguide integration for quantum photonic devices. Governmental agencies and international consortia recognize the strategic importance of quantum technologies. As a result, funding calls often encourage collaborative research that unites academic institutions, small and medium enterprises, large corporations, and research organizations. These programs can support the development of open-access foundries, shared design libraries, and standardized test protocols that make waveguide integration more accessible and reproducible.
However, policy support can sometimes lag behind technological needs. The absence of clearly defined standards for quantum photonic devices makes it difficult to compare results across groups or scale production. Creating recognized quality metrics, design kits, and benchmarking methodologies for waveguide integration would greatly assist the field. Policymakers could also mandate data sharing or open-source software development to accelerate learning and reduce duplication of effort. The ultimate goal is to create an environment where best practices emerge naturally, guided by community consensus and regulatory frameworks that reward reproducibility, transparency, and long-term thinking.
With the European Union, the United States, and parts of Asia all investing heavily in quantum technologies, there is an opportunity to learn from each other’s experiences and policy initiatives. International dialogues can identify pain points in waveguide integration and align funding priorities to fill gaps. By harmonizing approaches across regions, the field can move more quickly toward stable, scalable solutions that benefit the global community of quantum technology developers and users.
Looking Ahead: Opportunities for Breakthroughs
While the challenges presented here are significant, they are not insurmountable. The rapid pace of innovation in materials science, nanofabrication, and optical design tools suggests that many of the current limitations can be overcome with sustained effort and interdisciplinary thinking. If we consider the potential for fully integrated quantum computers on a chip, where complex photonic circuits implement quantum gates at scale and communicate with classical electronics seamlessly, waveguide integration becomes a linchpin technology. Achieving that vision may require revisiting fundamental assumptions about how waveguides are designed, fabricated, and tested.
Machine learning and artificial intelligence could guide the inverse design process, making waveguide structures more tolerant to fabrication uncertainties and environmental fluctuations. Novel materials, including two-dimensional semiconductors or topological photonics platforms, might reduce scattering losses or offer built-in isolation from environmental noise. Scaling up fabrication lines, establishing dedicated quantum photonic foundries, and implementing robust quality control systems can ensure that each device meets the exacting standards required for quantum information processing. Standardized packaging solutions, possibly leveraging advanced 3D printing or self-assembled optical components, could alleviate coupling losses and improve system stability.
However, these opportunities come with a warning: without a coherent strategy that ties together technological innovation, skill development, policy support, and global collaboration, the field risks fragmenting into isolated efforts. Each challenge—from waveguide design to PIC fabrication and optical packaging—must be addressed in concert. Solutions that only tackle one dimension of the problem in isolation may provide incremental improvements but fail to produce the transformative advances the quantum community seeks.
Conclusion and Call to Action
Waveguide integration for next-generation quantum photonic devices encapsulates a rich tapestry of challenges and opportunities. On the technical front, designers must contend with fabrication tolerances, material constraints, packaging intricacies, and the delicate balancing act required to preserve quantum coherence. On the organizational and cultural side, stakeholders must learn to communicate effectively, adopt standardized best practices, and embrace interdisciplinary training to ensure a skilled workforce. Policy measures and funding frameworks can either accelerate this progress by providing structured support and incentives for collaboration, or hinder it by failing to align research efforts with real-world requirements.
Addressing these challenges head-on requires a collective effort. Researchers, engineers, policymakers, and manufacturers must work in concert. Academic institutions and industry players need to establish structured training programs that equip new professionals with the necessary skills to navigate the complexity of PIC fabrication and optical packaging. Collaborative consortia, supported by governmental or transnational funding initiatives, can help create shared facilities and standardized libraries that reduce duplication of effort and uncertainty. In turn, these infrastructures can feed back into the research ecosystem, providing well-characterized platforms that enable advanced design methodologies and more reliable simulations.
As quantum photonic devices inch closer to industrial relevance, the community must remain realistic yet ambitious. Solutions to waveguide integration challenges will not appear overnight, nor will they emerge from a single breakthrough. Instead, incremental improvements, hard-earned through iterative refinement, critical self-assessment, and open dialogue, will gradually pave the way for robust, scalable quantum photonic technologies. By emphasizing challenges and focusing on evidence-based critiques, this review aims to spur that dialogue and encourage actionable steps toward a future where waveguide integration is no longer a barrier but an enabler of next-generation quantum photonic devices.
References and Further Reading
Agrell, E., Karlsson, M., & Chraplyvy, A. (2016). Roadmap of optical communications. J. Opt., 18(6), 063002. [https://doi.org/10.1088/2040-8978/18/6/063002]
Bogaerts, W., & Chrostowski, L. (2018). Photonic integrated circuit design automation: Past, present, and future. IEEE Nanotechnology Magazine, 12(2), 20–29. [https://doi.org/10.1109/MNANO.2018.2819409]
Flamini, F., Spagnolo, N., & Sciarrino, F. (2018). Photonic quantum information processing: a review. Rep. Prog. Phys., 82(1), 016001. [https://doi.org/10.1088/1361-6633/aad5b2]
Knill, E., Laflamme, R., & Milburn, G. J. (2001). A scheme for efficient quantum computation with linear optics. Nature, 409(6816), 46–52. [https://doi.org/10.1038/35051009]
Nielsen, M. A., & Chuang, I. L. (2010). Quantum Computation and Quantum Information: 10th Anniversary Edition. Cambridge University Press. [https://doi.org/10.1017/CBO9780511976667]
Perez, D., Gasulla, I., & Capmany, J. (2017). Field-programmable photonic arrays. Optics Express, 25(3), 282–294. [https://doi.org/10.1364/OE.25.000282]
Politi, A., Matthews, J. C. F., Thompson, M. G., & O’Brien, J. L. (2009). Integrated quantum photonics. IEEE Journal of Selected Topics in Quantum Electronics, 15(6), 1673–1684. [https://doi.org/10.1109/JSTQE.2009.2026060]
Soref, R. (2010). The past, present, and future of silicon photonics. IEEE Journal of Selected Topics in Quantum Electronics, 16(1), 167–176. [https://doi.org/10.1109/JSTQE.2009.2035196]
Spring, J. B., et al. (2013). On-chip low loss heralded source of pure single photons. Optics Express, 21(11), 13522–13532. [https://doi.org/10.1364/OE.21.013522]
Wang, J., Paesani, S., Ding, Y., Santagati, R., & Laing, A. (2018). Multidimensional quantum entanglement with large-scale integrated optics. Science, 360(6386), 285–291. [https://doi.org/10.1126/science.aar7053]
Xu, X., et al. (2020). Photonic inverse design: from fundamentals to applications. Adv. Opt. Photon., 12(3), 828–887. [https://doi.org/10.1364/AOP.381966]