This article explores the latest advancements in solar cell technology, focusing on improving energy conversion efficiency to meet global energy needs. It examines innovations in photovoltaic materials, such as perovskite solar cells, tandem structures, and organic photovoltaics, highlighting their potential to transform solar energy capture. The discussion also covers design improvements like Passivated Emitter and Rear Cell (PERC) architectures, heterojunction with intrinsic thin layer (HIT) technology, and bifacial solar cells, which enhance efficiency through advanced configurations. Additionally, the article reviews new manufacturing processes, including laser doping, automation, and sustainable practices, showing how the industry is optimizing performance while reducing environmental impact.
Case studies from Germany and Austria demonstrate the practical applications of these innovations, offering insights into successful projects and best practices. The article concludes with a look at future trends, such as next-generation materials, hybrid energy systems, and the role of policy and market forces, positioning solar technology as a key player in the renewable energy sector. This review provides both technical guidance and strategic insight, making it a valuable resource for engineers, researchers, and industry professionals working to advance high-efficiency solar cells.
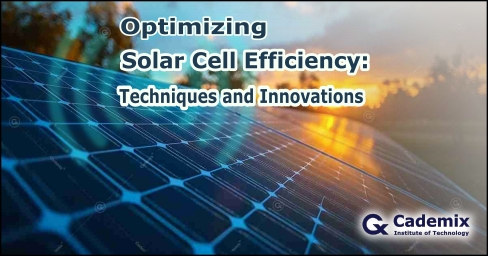
1.Introduction
Solar energy is rapidly becoming one of the most important sources of renewable energy in the world. As concerns about climate change and the depletion of fossil fuels grow, the need for clean, sustainable energy has never been more urgent. Solar cells, which convert sunlight into electricity, are at the heart of this transformation. Their efficiency—how well they convert solar energy into usable power—plays a crucial role in determining how much energy we can harvest from the sun.
Historically, the efficiency of solar cells has seen significant improvements, but challenges remain. Traditional silicon-based solar cells, while widely used, are approaching their theoretical efficiency limits. To meet the increasing energy demands and reduce the cost of solar energy, new materials and innovative designs are essential. The drive to improve efficiency is not just about producing more power; it’s also about making solar technology more viable for widespread adoption, particularly in regions with less sunlight or higher energy needs.
This article aims to explore the latest techniques and innovations that are pushing the boundaries of solar cell efficiency. We will delve into new materials, such as perovskites and tandem cells, that promise to exceed the performance of conventional silicon cells. We will also look at advanced designs and manufacturing processes that are making these technologies more practical and cost-effective. Throughout the discussion, we will reference examples from Germany and Austria, regions known for their strong commitment to renewable energy, to illustrate how these innovations are being applied in real-world settings.
By the end of this article, readers will gain a comprehensive understanding of the current state of solar cell technology, the challenges that remain, and the exciting future possibilities. Whether you are an engineer, a researcher, or an industry professional, this exploration of solar cell efficiency will provide valuable insights into the advancements that are shaping the future of energy.
2.Fundamental Concepts of Solar Cell Efficiency
Understanding the fundamentals of solar cell efficiency is crucial for grasping the innovations and techniques discussed later in this article. Efficiency in solar cells refers to the percentage of sunlight that a solar cell can convert into usable electricity. Several factors influence this efficiency, from the materials used in the cell to the physical processes that occur when sunlight hits the cell’s surface. In this section, we will cover the basic principles of solar cell operation, the key factors that affect efficiency, and the metrics used to measure this efficiency.
2.1 Basics of Solar Cell Operation
Solar cells operate based on the photovoltaic (PV) effect, a process where light energy is converted directly into electrical energy. When sunlight strikes the surface of a solar cell, it excites electrons in the cell’s material, usually a semiconductor like silicon. These excited electrons create an electric current as they move through the material. The movement of electrons generates direct current (DC) electricity, which can then be converted to alternating current (AC) for use in homes and businesses.
A typical solar cell consists of two layers of semiconductor material, one positively charged (p-type) and the other negatively charged (n-type). The junction between these two layers is where the magic happens. When photons from sunlight hit the cell, they knock electrons loose from atoms in the semiconductor. This creates electron-hole pairs—essentially, free electrons and the “holes” they leave behind. The internal electric field at the p-n junction drives these electrons and holes in opposite directions, creating a flow of electricity.
2.2 Key Factors Influencing Solar Cell Efficiency
Several factors influence how efficiently a solar cell can convert sunlight into electricity. Understanding these factors helps in identifying areas for improvement and innovation.
Bandgap Energy: The bandgap is a fundamental property of semiconductor materials. It represents the energy difference between the valence band (where electrons are normally found) and the conduction band (where electrons can move freely to conduct electricity). For a photon to free an electron and create electricity, its energy must be at least equal to the bandgap energy. If the bandgap is too large, many photons won’t have enough energy to free electrons. If it’s too small, the cell will lose energy as heat. Silicon, the most common material used in solar cells, has a bandgap that is well-suited to the spectrum of sunlight, making it highly efficient, but also limiting in terms of maximum efficiency potential.
Absorption of Sunlight: The ability of a solar cell to absorb light is critical. The more light that a cell can absorb, the more electricity it can generate. Different materials have different absorption efficiencies. For example, thin-film materials can absorb light more effectively than traditional silicon, which is why they are of interest for new types of solar cells. Surface texturing and anti-reflective coatings are also used to maximize light absorption by reducing the amount of light that is reflected away from the cell’s surface.
Charge Carrier Separation and Recombination: Once electron-hole pairs are created, they need to be separated and directed to the appropriate electrodes to generate electricity. The efficiency of this process depends on the quality of the semiconductor material and the design of the cell. Recombination, where electrons and holes recombine before they can be collected, is a major loss mechanism in solar cells. Innovations such as passivation layers, which reduce surface recombination, are crucial in improving efficiency.
External Factors: External conditions, such as temperature and the angle of sunlight incidence, also affect solar cell efficiency. Higher temperatures generally decrease the efficiency of solar cells because they increase the recombination rate of electrons and holes. The angle at which sunlight hits the cell can impact how much light is absorbed. Solar panels are often installed with adjustable mounts to optimize the angle of incidence throughout the year.
2.3 Metrics for Measuring Efficiency
Efficiency is a key metric in the performance of solar cells, but it is not the only one. Several parameters are used to evaluate and compare solar cells.
Fill Factor (FF): The fill factor is a measure of a solar cell’s quality. It is the ratio of the actual maximum obtainable power to the theoretical power (if both current and voltage were at their maximum values at the same time). A higher fill factor indicates a better quality solar cell with fewer losses. It is typically between 0.7 and 0.85 for good quality cells.
Open-Circuit Voltage (Voc): This is the maximum voltage available from a solar cell when it is not connected to an external circuit (i.e., no current is flowing). The open-circuit voltage is influenced by the cell’s material and design. Higher open-circuit voltages contribute to higher efficiency, but they are also limited by the material properties, particularly the bandgap.
Short-Circuit Current (Isc): This is the maximum current that flows through the solar cell when the terminals are shorted. It is directly proportional to the amount of light absorbed by the cell. Factors such as the cell’s surface area, light absorption efficiency, and the number of photons hitting the cell determine the short-circuit current.
Energy Conversion Efficiency (η): This is the ratio of the electrical power output to the power input from sunlight, typically expressed as a percentage. It is the most common measure of a solar cell’s performance. For example, a solar cell with a 20% efficiency converts 20% of the incoming solar energy into electricity, while the rest is lost as heat or reflected light.
The current efficiency benchmarks for different solar cell technologies vary. Conventional silicon-based cells typically achieve efficiencies around 15-22%, while newer technologies like perovskite cells are reaching efficiencies above 25% in laboratory settings. Tandem cells, which combine different materials to capture more of the solar spectrum, have achieved efficiencies above 30%.
In summary, the efficiency of a solar cell is influenced by various factors, including material properties, design, and external conditions. By understanding these fundamentals, we can better appreciate the innovations that aim to overcome existing limitations and push the boundaries of what solar cells can achieve. The next sections of this article will delve into these innovations, exploring how new materials, designs, and manufacturing processes are driving the quest for higher efficiency in solar energy.
3.Material Innovations for Higher Efficiency
Advancements in material science are crucial for improving the efficiency of solar cells. Traditional silicon-based solar cells have dominated the market for decades, but their efficiency is approaching a theoretical limit, known as the Shockley-Queisser limit, which caps the maximum efficiency at about 33% for single-junction cells. To break through this barrier, researchers are exploring new materials that can absorb more sunlight, convert energy more efficiently, and be produced at lower costs. In this section, we will examine the most promising material innovations, including perovskite solar cells, tandem solar cells, and organic photovoltaics, all of which are driving significant efficiency gains in solar technology.
3.1 Emerging Photovoltaic Materials
3.1.1 Perovskite Solar Cells
Perovskite solar cells have emerged as one of the most exciting developments in the field of photovoltaics. The term “perovskite” refers to a specific crystal structure, not a single material. Most commonly, these cells are made from a compound called methylammonium lead halide. What makes perovskites so appealing is their ability to absorb a broad spectrum of light, including low-energy photons that traditional silicon cells cannot efficiently capture.
Perovskite solar cells have shown rapid efficiency improvements, reaching over 25% in laboratory settings in just a few years of development. This is comparable to the best silicon-based cells, but with the added advantage of potentially lower manufacturing costs. The materials can be processed using simple, low-temperature techniques, such as spin-coating, which reduces the overall cost of production.
However, challenges remain. One significant issue is stability. Perovskite materials are sensitive to moisture, heat, and UV light, which can degrade the cell’s performance over time. Researchers are working on developing encapsulation techniques and more stable perovskite compounds to overcome these issues.
In Germany, institutions like the Fraunhofer Institute for Solar Energy Systems (ISE) are at the forefront of perovskite research, exploring ways to integrate these cells into existing silicon-based modules, creating hybrid or tandem cells that leverage the strengths of both materials.
3.1.2 Tandem Solar Cells
Tandem solar cells represent another significant leap in solar technology. These cells stack two or more different photovoltaic materials, each tuned to absorb different parts of the solar spectrum. By capturing more of the sun’s energy, tandem cells can achieve efficiencies beyond the Shockley-Queisser limit of single-junction cells.
One common approach is to pair a traditional silicon cell with a perovskite top cell. The perovskite layer captures high-energy photons, while the silicon layer absorbs the lower-energy photons. This combination has led to tandem cells with efficiencies exceeding 30% in laboratory conditions.
The fabrication of tandem cells involves carefully matching the two materials’ bandgaps to ensure optimal light absorption and minimize energy losses. The challenge lies in ensuring that the two materials work together efficiently without introducing significant complexity or cost into the manufacturing process.
In Austria, the Technical University of Vienna (TU Wien) has been actively researching tandem solar cells, focusing on optimizing the interface between different materials to improve efficiency and durability. These efforts are crucial for bringing tandem cells from the lab to the market, where they can offer a higher-performance alternative to traditional solar panels.
3.1.3 Organic Photovoltaics (OPV)
Organic photovoltaics (OPV) are another promising material innovation in the solar industry. Unlike silicon or perovskite cells, OPVs are made from organic compounds, primarily carbon-based polymers. These materials can be processed at low temperatures and deposited on flexible substrates, making OPVs lightweight, flexible, and potentially very inexpensive to produce.
OPVs typically have lower efficiencies compared to silicon or perovskite cells, with current records around 17%. However, their unique properties make them attractive for specific applications, such as portable solar chargers, wearable devices, or building-integrated photovoltaics (BIPV), where flexibility and aesthetics are more important than maximum efficiency.
The low cost of OPV production is one of its major advantages. The materials can be printed using roll-to-roll processes, similar to how newspapers are printed, which could lead to large-scale, inexpensive solar panels. Additionally, OPVs can be made semi-transparent, allowing them to be integrated into windows or other building surfaces.
Germany has been a leader in OPV research, with institutions like the Karlsruhe Institute of Technology (KIT) developing new organic materials and exploring innovative applications for this technology. While OPVs may not replace silicon or perovskite cells in terms of efficiency, they offer unique advantages that could complement existing solar technologies.
3.2 Advanced Coatings and Texturing Techniques
In addition to exploring new photovoltaic materials, researchers and engineers are also developing advanced coatings and texturing techniques to enhance the efficiency of solar cells. These methods focus on maximizing light absorption and minimizing losses due to reflection or recombination.
3.2.1 Anti-Reflective Coatings
One of the simplest yet most effective ways to improve solar cell efficiency is to apply an anti-reflective coating (ARC) to the cell’s surface. Without an ARC, a significant portion of sunlight is reflected off the surface of the solar cell and lost. By reducing this reflection, more light is absorbed, and thus, more electricity is generated.
ARCs are typically made from thin layers of materials like silicon nitride or titanium dioxide, which have a refractive index that matches the solar cell’s material. These coatings can reduce reflection losses to less than 5%. Additionally, advanced ARCs can be designed to work across a broad range of wavelengths and angles of incidence, ensuring maximum light absorption throughout the day and year.
In Germany, several companies and research institutes are working on developing multi-layer ARCs that not only reduce reflection but also enhance the durability of solar cells, protecting them from environmental degradation.
3.2.2 Surface Texturing
Surface texturing is another technique used to enhance light absorption in solar cells. By creating a textured surface, such as a grid of tiny pyramids or a pattern of microgrooves, the path of incoming light is altered, increasing the likelihood that the light will be absorbed rather than reflected.
Texturing can be applied directly to the semiconductor material during the manufacturing process or as a separate layer. The textured surface effectively traps light within the solar cell, increasing the amount of light absorbed and, consequently, the amount of electricity generated.
In the European market, several solar manufacturers are adopting these texturing techniques in their production lines. For example, some companies in Austria are using nanotechnology to create finely textured surfaces that improve light trapping and increase the overall efficiency of their solar panels.
Conclusion of Section 3
Material innovations in solar cell technology are pushing the boundaries of efficiency and making solar energy more accessible and affordable. From the rapid development of perovskite and tandem solar cells to the unique advantages of organic photovoltaics, these new materials offer exciting possibilities for the future of solar power. Additionally, advancements in coatings and texturing techniques are further enhancing the performance of these materials, ensuring that more sunlight is captured and converted into electricity.
As we move forward, the combination of these material innovations with advanced designs and manufacturing processes, discussed in the next sections, will play a critical role in achieving the high-efficiency solar cells needed to meet global energy demands and reduce our reliance on fossil fuels.
4.Design Innovations Enhancing Efficiency
Advancements in material science have significantly improved solar cell efficiency, but innovative designs are equally crucial. By reimagining how solar cells are structured and operate, engineers can extract more energy from the same amount of sunlight. This section explores several design innovations that have made substantial contributions to enhancing solar cell efficiency. We will delve into advanced cell architectures like Passivated Emitter and Rear Cell (PERC) and Heterojunction with Intrinsic Thin Layer (HIT) technologies, the concept of Concentrated Photovoltaics (CPV), and the benefits of bifacial solar cells.
4.1 Advanced Cell Architectures
4.1.1 Passivated Emitter and Rear Cell (PERC)
The Passivated Emitter and Rear Cell (PERC) technology represents a significant leap in solar cell design. Traditional solar cells often suffer from efficiency losses due to electron recombination at the cell’s surfaces. PERC addresses this issue by introducing passivation layers that reduce these losses, leading to higher efficiency.
Working Principle and Efficiency Gains
In a standard solar cell, the rear surface can be a site of electron recombination, where electrons recombine with holes before contributing to the electric current. PERC technology adds a dielectric passivation layer to the rear surface, minimizing these recombination events. Additionally, small openings in this layer allow for local contacts, ensuring efficient charge collection.
This design not only reduces recombination but also reflects unabsorbed light back into the silicon layer, giving photons a second chance to generate electron-hole pairs. The result is an increase in both voltage and current, leading to higher overall efficiency. PERC cells have demonstrated efficiency gains of about 1% absolute over traditional cells, which is substantial in the solar industry.
Adoption in Commercial Products
Given its compatibility with existing manufacturing processes, PERC has seen rapid adoption in the commercial sector. Manufacturers can often upgrade their production lines to incorporate PERC technology without significant overhauls. Many leading solar companies now offer PERC-based panels, boasting efficiencies exceeding 22%. This widespread adoption underscores PERC’s practicality and effectiveness in enhancing solar cell performance.
4.1.2 Heterojunction with Intrinsic Thin Layer (HIT)
Another innovative architecture is the Heterojunction with Intrinsic Thin Layer (HIT) technology. Developed initially by Sanyo (now part of Panasonic), HIT combines crystalline silicon with thin layers of amorphous silicon to harness the advantages of both materials.
Explanation of HIT Technology
In HIT cells, a crystalline silicon wafer serves as the core, providing a high-quality substrate for charge collection. Thin layers of intrinsic (undoped) amorphous silicon are deposited on both sides of this wafer, acting as passivation layers to reduce surface recombination. These are followed by doped amorphous silicon layers that form the p-n junctions necessary for photovoltaic action.
This combination results in several benefits:
- High Efficiency: HIT cells have achieved efficiencies above 24% in laboratory settings, with commercial products reaching around 22%.
- Low Temperature Coefficient: These cells maintain performance better at higher temperatures compared to traditional cells, making them suitable for various climates.
- Bifacial Capability: The symmetrical structure allows for light absorption from both sides, increasing potential energy generation.
Case Studies from Manufacturers in Germany and Austria
In Germany, companies like Meyer Burger have been instrumental in advancing HIT technology. They have developed production equipment that enables efficient fabrication of HIT cells, contributing to their commercial viability. Austrian manufacturers, while smaller in scale, have also explored HIT technology, recognizing its potential for high-performance solar solutions. These efforts demonstrate a commitment to integrating cutting-edge designs into the European solar industry.
4.2 Concentrated Photovoltaics (CPV)
Concentrated Photovoltaics (CPV) offer a different approach to solar energy capture by using optical components to focus sunlight onto high-efficiency solar cells.
4.2.1 Concept and Benefits
Use of Lenses or Mirrors to Focus Sunlight
CPV systems employ lenses or mirrors to concentrate sunlight, often by factors of 2 to over 1,000, onto small, high-performance photovoltaic cells. By concentrating light, CPV reduces the amount of photovoltaic material needed, allowing the use of more efficient, but typically more expensive, materials like multi-junction cells.
Efficiency Potential of CPV Systems
Multi-junction cells used in CPV can achieve efficiencies exceeding 40% in laboratory conditions. Even with system-level losses, CPV installations can surpass 30% efficiency, outperforming traditional photovoltaic systems. The concentrated light allows these high-efficiency cells to operate at their optimal point, maximizing energy generation.
4.2.2 Applications and Case Studies
Deployment in High-Irradiance Regions
CPV systems are best suited for regions with high direct sunlight, as they rely on direct beams rather than diffuse light. Therefore, their deployment is more common in areas like Southern Europe, North Africa, and the southwestern United States.
Examples of CPV Projects in Europe
While Germany and Austria have less favorable climates for CPV, European projects have demonstrated its potential. For instance, the Solar Tec International plant in Spain utilizes CPV technology to harness the region’s abundant sunlight. These installations showcase CPV’s capability to provide high-efficiency solar power in suitable environments.
However, CPV systems require precise solar tracking mechanisms to maintain focus on the sun, adding complexity and cost. They also perform poorly under cloudy conditions, limiting their applicability. Despite these challenges, CPV remains a compelling option in specific contexts.
4.3 Bifacial Solar Cells
Bifacial solar cells represent a design innovation that allows for energy capture from both sides of the panel, increasing total energy generation.
4.3.1 Dual-Side Energy Capture
Description of Bifacial Cell Technology
Traditional solar panels are monofacial, absorbing light only from the front side. Bifacial cells, in contrast, are designed to capture light from both the front and rear. The rear side can absorb light reflected from the ground or surrounding surfaces, known as albedo light.
This design involves using transparent or reflective backsheets and optimizing cell placement to avoid shading. By harnessing additional light, bifacial panels can produce more energy without increasing the panel’s footprint.
Efficiency Improvements from Capturing Reflected Light
The efficiency gains from bifacial panels depend on various factors, including ground reflectivity, panel height, and installation angle. In optimal conditions, such as installations over snow or white surfaces, bifacial panels can generate up to 30% more energy compared to their monofacial counterparts. Even in standard conditions, a 10-15% increase is common.
4.3.2 Practical Implementations
Deployment Examples in Germany and Austria
Germany has seen several bifacial solar installations, capitalizing on limited space to maximize energy yield. For example, the Herne solar farm incorporates bifacial panels to enhance production. In Austria, the alpine environment offers high albedo conditions during winter months due to snow cover, making bifacial technology particularly advantageous.
Performance Comparisons with Traditional Solar Cells
Studies comparing bifacial and monofacial installations have consistently shown the former’s superiority in energy generation. While bifacial panels may have higher initial costs, the increased energy yield can lead to better long-term economic returns. This balance makes bifacial technology an attractive option for both utility-scale and residential projects.
Conclusion of Section 4
Design innovations like PERC and HIT architectures, CPV systems, and bifacial cells have significantly contributed to enhancing solar cell efficiency. By addressing various loss mechanisms and optimizing energy capture, these designs push the boundaries of what’s possible in photovoltaic technology. As the solar industry continues to evolve, integrating such innovative designs will be key to meeting global energy demands sustainably.
5.Manufacturing Process Innovations
In the quest to improve solar cell efficiency, innovations in manufacturing processes are just as important as advancements in materials and design. The way solar cells are produced can significantly impact their performance, cost, and environmental footprint. This section explores key innovations in manufacturing techniques, focusing on high-efficiency production processes, the integration of automation and artificial intelligence (AI), and the drive for sustainability in solar cell manufacturing. These innovations are helping to make solar energy more accessible, affordable, and environmentally friendly.
5.1 High-Efficiency Production Techniques
As the demand for more efficient solar cells grows, so does the need for advanced manufacturing techniques that can produce high-performance cells at scale. Several production methods have been developed to enhance the efficiency of solar cells while keeping costs competitive.
5.1.1 Laser Doping
Laser doping is a cutting-edge technique used to improve the efficiency of solar cells by precisely introducing dopants into specific regions of the cell. Dopants are impurities added to a semiconductor to change its electrical properties, and in solar cells, they help to form the p-n junction that is critical for generating electricity.
Explanation of Laser Doping and Its Impact on Efficiency
In traditional doping methods, dopants are diffused throughout the semiconductor material, which can lead to uneven distribution and efficiency losses. Laser doping, however, allows for precise control over where and how much dopant is added. A laser beam is used to locally melt the surface of the semiconductor, allowing dopants to diffuse more effectively into the desired areas. This results in better-defined p-n junctions, reduced recombination losses, and ultimately, higher efficiency.
Laser doping is particularly effective in creating selective emitters, where different regions of the cell are doped to different levels, optimizing the cell’s response to varying light conditions. This technique is commonly used in the production of Passivated Emitter and Rear Cell (PERC) solar cells, contributing to their superior performance.
Industry Examples of Laser Doping in Solar Cell Manufacturing
Many leading solar manufacturers, including those in Germany, have adopted laser doping in their production processes. For example, the Fraunhofer Institute for Solar Energy Systems (ISE) has been instrumental in developing and refining laser doping techniques, helping to push the efficiency of commercial solar cells to new heights.
5.1.2 Advanced Wafer Technologies
The production of silicon wafers—the thin slices of semiconductor material that form the basis of most solar cells—has also seen significant advancements. Two key innovations in this area are the development of thinner wafers and kerfless wafer production.
Thin Wafers and Kerfless Wafer Production
Traditional silicon wafers are typically around 180-200 micrometers thick, but advances in slicing technology have allowed manufacturers to produce thinner wafers, sometimes as thin as 100 micrometers. Thinner wafers use less silicon, reducing material costs and energy consumption during production. However, thinner wafers are also more fragile, so innovations in handling and processing are necessary to prevent breakage.
Kerfless wafer production is another exciting development. Traditional wafer slicing involves cutting silicon ingots with a wire saw, which generates significant material waste in the form of silicon dust (kerf). Kerfless production methods, such as epitaxial growth or lift-off techniques, create wafers directly from a substrate without cutting, virtually eliminating waste. This not only reduces costs but also minimizes the environmental impact of wafer production.
Efficiency Benefits and Cost Reduction
Both thin and kerfless wafers contribute to higher efficiency by reducing the amount of silicon used without sacrificing performance. They also lower the overall cost of solar cells, making solar energy more competitive with other forms of energy. German companies like NexWafe are pioneering kerfless wafer production, aiming to bring this technology to commercial scale in the near future.
5.2 Automation and AI in Solar Cell Manufacturing
Automation and artificial intelligence (AI) are transforming solar cell manufacturing, making the production process faster, more precise, and less prone to errors. These technologies are helping manufacturers achieve higher efficiency and lower costs while maintaining high-quality standards.
5.2.1 Role of Automation
Reducing Defects and Improving Precision
Automation plays a crucial role in modern solar cell manufacturing by reducing human error and ensuring consistent quality. Automated systems can precisely control every aspect of the production process, from wafer slicing and cell assembly to testing and packaging. This precision helps reduce defects, such as microcracks in wafers or misaligned cell components, which can negatively impact efficiency.
Automated production lines also enable manufacturers to scale up production without compromising on quality. For example, robotic arms can assemble solar panels with millimeter precision, ensuring that each cell is placed correctly and that electrical connections are flawless. This level of precision is essential for achieving the high efficiencies demanded by the market.
Examples of Automated Production Lines in Germany
Germany has been at the forefront of integrating automation into solar manufacturing. Companies like Meyer Burger have developed fully automated production lines that significantly reduce production costs while improving the quality and efficiency of solar cells. These automated systems are capable of producing large volumes of high-efficiency solar cells with minimal human intervention.
5.2.2 AI and Machine Learning
Applications in Quality Control and Process Optimization
Artificial intelligence (AI) and machine learning are becoming increasingly important in optimizing solar cell manufacturing. These technologies can analyze vast amounts of data from the production process to identify patterns and correlations that might not be immediately apparent to human operators. This information can then be used to fine-tune production parameters in real-time, leading to continuous improvements in efficiency and yield.
For example, AI can be used to predict and prevent defects by analyzing images of wafers or cells for early signs of damage or misalignment. Machine learning algorithms can also optimize the doping process, adjusting laser settings to achieve the best possible p-n junctions based on previous production data.
AI is also being used to streamline the supply chain, ensuring that materials are delivered just in time and that production schedules are optimized for maximum efficiency. These innovations help manufacturers reduce costs and increase output, making solar energy more accessible to a broader market.
Case Studies of AI Integration in European Solar Cell Production
Several European solar manufacturers are leading the way in AI integration. In Germany, companies like Siemens are leveraging AI to enhance both the efficiency and reliability of their solar production lines. These AI-driven systems are capable of learning from each production run, continuously improving the quality and efficiency of the cells produced.
5.3 Sustainability in Manufacturing
As the solar industry grows, there is increasing emphasis on making the manufacturing process more sustainable. This involves reducing the environmental impact of production, improving the recyclability of solar panels, and developing new ways to reuse materials.
5.3.1 Green Manufacturing Practices
Reducing Environmental Impact During Production
Green manufacturing practices aim to minimize the environmental footprint of solar cell production. This includes reducing energy consumption, minimizing waste, and using non-toxic materials. For instance, some manufacturers are switching to renewable energy sources to power their production facilities, significantly lowering their carbon footprint.
Water use is another area of focus. Traditional solar manufacturing processes consume large amounts of water, particularly for cooling and cleaning wafers. Innovations in water recycling and purification are helping to reduce this demand, making the production process more sustainable.
Circular Economy Approaches in Solar Manufacturing
The concept of a circular economy—where products are designed for durability, reuse, and recyclability—is gaining traction in the solar industry. This approach seeks to minimize waste and extend the lifecycle of materials used in solar cells.
One example of a circular economy approach is the development of modules that can be easily disassembled at the end of their life, allowing valuable materials like silicon, silver, and copper to be recovered and reused. This not only reduces the environmental impact of solar panel disposal but also lowers the demand for raw materials.
5.3.2 Recycling and Reuse of Solar Cell Materials
Techniques for Recovering Valuable Materials
Recycling solar panels at the end of their lifecycle is becoming increasingly important as the installed base of solar panels grows. Innovative recycling techniques are being developed to recover valuable materials from old panels, including silicon, glass, and metals like silver and copper.
One promising method is thermal treatment, where panels are heated to break down the bonding materials, allowing the individual components to be separated and recovered. Chemical treatments are also being explored to dissolve and recover specific materials, particularly metals.
Examples from German and Austrian Companies
Several companies in Germany and Austria are leading the way in solar panel recycling. For example, the German company Reiling has developed a process for recycling silicon from old panels, which can then be used in new solar cells. In Austria, research institutions are exploring ways to improve the recyclability of solar panels, ensuring that the materials used today can be part of tomorrow’s energy solutions.
Innovations in manufacturing processes are crucial for the continued improvement of solar cell efficiency and the reduction of costs. Techniques like laser doping, advanced wafer production, automation, and AI are making it possible to produce high-performance solar cells more efficiently and sustainably than ever before. At the same time, a focus on green manufacturing practices and recycling ensures that the solar industry can grow without compromising the environment. As these innovations continue to evolve, they will play a key role in making solar energy a dominant force in the global energy landscape.
6.Case Studies and Applications
The practical application of advanced solar technologies is crucial for understanding their true impact and potential. By examining successful implementations, we can see how these innovations perform in real-world scenarios, providing insights into their benefits and challenges. This section presents case studies from Germany and Austria, showcasing how high-efficiency solar cells and innovative designs have been applied effectively. These examples highlight the role of cutting-edge solar technology in meeting energy needs while supporting sustainability goals.
6.1 Successful Implementations of High-Efficiency Solar Cells
High-efficiency solar cells have been integrated into various projects worldwide, demonstrating their effectiveness and versatility. The following case studies illustrate how these technologies have been deployed in Germany and Austria, resulting in significant energy production gains and cost savings.
Germany: The Herne Solar Farm
The Herne Solar Farm, located in North Rhine-Westphalia, Germany, is an exemplary project that showcases the potential of advanced solar technology on a large scale. This project combines bifacial solar panels with Passivated Emitter and Rear Cell (PERC) technology to maximize energy generation.
Project Overview
The Herne Solar Farm is built on a repurposed coal mining site, reflecting Germany’s transition from traditional energy sources to renewable energy. With an installed capacity of 10 megawatts (MW), the farm provides clean energy to thousands of homes, contributing to the region’s sustainable energy goals.
Technology and Performance
The project uses bifacial PERC panels, which capture sunlight from both the front and rear sides. This design increases overall energy production by about 15% compared to traditional monofacial panels. The farm’s location, with reflective ground surfaces, further enhances the performance of these bifacial panels.
Additionally, the farm employs an advanced tracking system that adjusts the angle of the panels throughout the day, optimizing their exposure to sunlight. This combination of high-efficiency panels and tracking technology has boosted the farm’s energy output by approximately 20% compared to fixed-tilt installations using standard panels.
Lessons Learned
The Herne Solar Farm demonstrates that high-efficiency solar technologies can significantly enhance energy production, even in regions with moderate sunlight. The project highlights the importance of integrating complementary technologies, such as tracking systems, to fully leverage the capabilities of advanced solar cells.
Austria: Vienna’s Urban Solar Initiative
In Austria, the Vienna Urban Solar Initiative represents a pioneering effort to incorporate high-efficiency solar technology into an urban environment. This initiative focuses on addressing the challenges of limited space and the need for aesthetically pleasing designs in densely populated areas.
Project Overview
The Vienna Urban Solar Initiative is part of the city’s broader strategy to become carbon-neutral by 2040. The project involves installing high-efficiency solar panels on various public, residential, and commercial buildings across the city, integrating solar energy into the urban fabric.
Technology and Performance
The project primarily utilizes Heterojunction with Intrinsic Thin Layer (HIT) solar panels, which are known for their high efficiency and excellent performance under low-light conditions. These panels are particularly effective in urban settings, where shading from buildings can reduce sunlight exposure.
A key feature of this initiative is the use of Building-Integrated Photovoltaics (BIPV). In several new constructions, solar panels are integrated directly into the building materials, such as facades and rooftops, blending functionality with aesthetics. These BIPV systems not only generate electricity but also enhance the visual appeal of the buildings, demonstrating that solar technology can be both efficient and architecturally attractive.
The combination of HIT panels and BIPV has enabled the Vienna Urban Solar Initiative to achieve higher energy yields in limited spaces, making solar energy more viable in urban environments. The project has also helped reduce the city’s reliance on external energy sources, increasing its energy independence.
Lessons Learned
The Vienna Urban Solar Initiative illustrates how high-efficiency solar technologies can be successfully integrated into urban environments. The use of HIT panels and BIPV provides a model for other cities seeking to expand their solar capacity without sacrificing aesthetics or space. This initiative underscores the importance of innovation in overcoming the unique challenges posed by urban settings.
6.2 Examples from Germany and Austria
Germany and Austria have been at the forefront of adopting and implementing advanced solar technologies. The following examples highlight how these countries are applying high-efficiency solar cells in different contexts, contributing to their renewable energy goals.
Germany: The Solar Factory of the Future
Germany’s Solar Factory of the Future, led by the Fraunhofer Institute for Solar Energy Systems (ISE), exemplifies the potential of integrating advanced manufacturing techniques with high-efficiency solar technologies. This project focuses on creating a fully automated, AI-driven production facility that leverages the latest innovations in solar cell manufacturing.
Project Overview
The Solar Factory of the Future aims to reduce production costs while enhancing the efficiency and sustainability of solar cells. The factory is designed to operate with minimal human intervention, using AI to optimize production processes in real time. It also incorporates green manufacturing practices, such as utilizing renewable energy sources to power the facility and recycling water used in production.
Technology and Performance
The factory produces next-generation solar cells, including tandem and perovskite cells. By integrating advanced manufacturing techniques like laser doping and kerfless wafer production, the factory has achieved solar cell efficiencies exceeding 25%. The use of AI in the production process has significantly reduced defects, improving both yield and quality.
Lessons Learned
The Solar Factory of the Future demonstrates the importance of innovation in manufacturing for driving the solar industry forward. By combining AI, advanced production techniques, and sustainability practices, this project showcases how the future of solar manufacturing can achieve both efficiency and environmental responsibility.
Austria: The Gleisdorf Solar Roof Initiative
Austria’s Gleisdorf Solar Roof Initiative is another example of successful solar technology integration. This initiative focuses on deploying high-efficiency solar panels on rooftops throughout the town of Gleisdorf, enhancing local energy production and reducing dependence on external power sources.
Project Overview
The Gleisdorf Solar Roof Initiative is part of a broader effort to increase the use of renewable energy in small towns and rural areas. The project involves installing high-efficiency solar panels on residential, commercial, and public buildings across Gleisdorf, with a focus on maximizing energy production in limited spaces.
Technology and Performance
The project uses advanced PERC and bifacial panels to maximize energy capture. The panels are installed on rooftops with varying orientations, and the use of bifacial technology helps capture reflected sunlight, further boosting energy production.
In addition to the panels, the initiative includes an energy management system that optimizes the distribution and use of solar energy within the town. This system ensures that solar power is used efficiently, reducing reliance on the grid during peak production times.
Lessons Learned
The Gleisdorf Solar Roof Initiative highlights the potential of high-efficiency solar technology in smaller communities. By using advanced panels and energy management systems, the project demonstrates how towns and rural areas can increase their energy independence and contribute to national renewable energy goals.
These case studies from Germany and Austria illustrate the practical application and benefits of high-efficiency solar technologies in various settings, from large-scale solar farms to urban and rural initiatives. By leveraging advanced materials, designs, and manufacturing processes, these projects have achieved significant gains in energy production and sustainability. The lessons learned from these implementations provide valuable insights for future projects, helping to guide the continued growth and development of the solar industry worldwide.
7.Future Directions and Trends
The solar industry is rapidly evolving, driven by the urgent need for sustainable energy solutions and the continuous push for higher efficiency and lower costs. As solar technology matures, new materials, designs, and manufacturing processes are emerging, promising to revolutionize the way we harness and use solar energy. This section explores the future directions and trends in solar technology, focusing on next-generation materials, the integration of solar energy with other technologies, and the role of policy and market dynamics in shaping the future of the industry.
7.1 Next-Generation Materials and Technologies
One of the most exciting areas of development in the solar industry is the exploration of new materials and technologies that could dramatically increase the efficiency of solar cells beyond what is currently possible with silicon-based technology.
Quantum Dot Solar Cells
Quantum dots are nanoscale semiconductor particles that have unique optical and electronic properties due to their size. These properties can be tuned by changing the size of the quantum dots, allowing them to absorb different parts of the solar spectrum more efficiently than traditional materials.
Potential and Challenges
Quantum dot solar cells have the potential to achieve very high efficiencies, possibly exceeding 30%, by capturing a broader range of the solar spectrum. They can be used in tandem with other materials, like perovskites or silicon, to create multi-junction cells that maximize light absorption.
However, challenges remain in terms of stability, scalability, and cost. Quantum dots are still primarily in the research phase, and significant work is needed to bring them to commercial viability. Researchers are focusing on improving the stability of quantum dot materials and finding ways to produce them at scale without compromising their performance.
Multi-Junction Solar Cells
Multi-junction solar cells stack several layers of different materials, each tuned to absorb a specific part of the solar spectrum. This design allows them to achieve efficiencies far beyond those of single-junction cells.
Advancements and Applications
Recent advancements in multi-junction cells have pushed efficiencies close to 50% in laboratory settings. These cells are particularly well-suited for applications where space is limited, and efficiency is paramount, such as in space-based solar power systems or concentrated photovoltaics (CPV).
While multi-junction cells are currently more expensive to produce than conventional cells, ongoing research and development are focused on reducing costs and improving their scalability. As manufacturing techniques improve, multi-junction cells could become more widely used in both terrestrial and extraterrestrial applications.
7.2 Integration with Other Technologies
As solar technology advances, its integration with other technologies is becoming increasingly important. This integration can enhance the efficiency and utility of solar energy, making it a more versatile and reliable source of power.
Hybrid Systems Combining Solar with Energy Storage
One of the most significant trends in the solar industry is the integration of solar power with energy storage systems, such as batteries. This combination allows for the storage of excess energy generated during the day for use at night or during periods of low sunlight, addressing one of the main limitations of solar power—its intermittency.
Benefits and Challenges
Hybrid solar and storage systems can provide a more consistent and reliable energy supply, reduce dependence on the grid, and increase energy independence. They are particularly valuable in remote or off-grid locations where access to consistent power is essential.
The main challenges of hybrid systems are related to the cost and lifespan of the batteries. However, advancements in battery technology, such as the development of more efficient and durable lithium-ion batteries and the exploration of alternative storage methods like flow batteries, are helping to overcome these obstacles.
Smart Grids and Solar Energy
The concept of smart grids—electricity networks that use digital technology to monitor and manage the flow of electricity—offers another avenue for integrating solar power more effectively into the energy system.
How Smart Grids Enhance Solar Energy Use
Smart grids can dynamically balance supply and demand, integrate a higher proportion of renewable energy sources, and improve the reliability and efficiency of electricity distribution. By incorporating real-time data and analytics, smart grids can optimize the use of solar power, reduce waste, and better accommodate the variable nature of solar energy.
In Germany, the Energiewende (energy transition) initiative is a prime example of how smart grid technology is being used to integrate large amounts of renewable energy, including solar, into the national grid. This integration is essential for achieving the country’s ambitious renewable energy targets.
7.3 Policy and Market Trends
The future of solar energy is also heavily influenced by policy and market dynamics. Government policies, incentives, and market conditions play a crucial role in shaping the direction of the solar industry, determining the pace of adoption and the types of technologies that become mainstream.
Government Policies and Incentives
Government policies and incentives have been instrumental in driving the growth of the solar industry. Subsidies, tax credits, feed-in tariffs, and renewable energy mandates have all contributed to the widespread adoption of solar power.
Trends in Policy Support
In Europe, particularly in countries like Germany and Austria, there has been strong governmental support for solar energy through various incentive programs. However, as solar technology matures and becomes more cost-competitive, there is a shift towards reducing subsidies and focusing on creating a more competitive market environment.
This transition poses both opportunities and challenges. On the one hand, it encourages innovation and cost reductions; on the other hand, it may slow down the deployment of new technologies that are still in the early stages of development. The future of solar energy policy will likely involve a balance between continuing to support emerging technologies and fostering a competitive market.
Market Dynamics and Global Competition
The global solar market is becoming increasingly competitive, with countries like China, the United States, and India playing significant roles in the production and deployment of solar technology. Europe, traditionally a leader in renewable energy, is facing increased competition from these emerging markets.
Impact on Innovation and Adoption
This global competition is driving innovation, as companies and countries strive to gain a competitive edge through technological advancements and cost reductions. It also affects the adoption of new technologies, as companies must balance the need for innovation with the pressures of competing in a global market.
For European companies, maintaining leadership in solar technology will require continued investment in research and development, as well as strategic partnerships and collaborations. Germany and Austria, with their strong research institutions and supportive policy environments, are well-positioned to remain at the forefront of solar innovation, but they will need to adapt to the changing global landscape.
The future of solar energy is bright, with numerous innovations and trends poised to transform the industry. Next-generation materials like quantum dots and multi-junction cells promise to push the boundaries of efficiency, while the integration of solar power with energy storage and smart grid technologies will enhance its reliability and versatility. At the same time, government policies and global market dynamics will play a critical role in shaping the direction of the industry.
As we look ahead, it is clear that the continued evolution of solar technology will be essential for meeting global energy demands and addressing the challenges of climate change. By staying at the forefront of innovation and adapting to changing market conditions, the solar industry can continue to grow and thrive, providing clean, renewable energy for a sustainable future.
8.Conclusion
The solar energy industry is on the cusp of a major transformation, driven by rapid advancements in technology and a growing global commitment to renewable energy. Throughout this article, we have explored the key innovations and techniques that are shaping the future of solar power, from new materials and cutting-edge designs to advanced manufacturing processes and integrated systems. As we conclude, it is important to reflect on the significance of these developments and consider the path forward for the solar industry.
8.1 Summary of Key Points
In recent years, significant progress has been made in enhancing the efficiency of solar cells. Material innovations, such as perovskite solar cells, tandem cells, and quantum dots, are pushing the boundaries of what is possible, offering the potential for much higher energy conversion rates than traditional silicon-based cells. These materials are not only more efficient but also have the potential to reduce costs and make solar energy more accessible globally.
Design innovations like Passivated Emitter and Rear Cell (PERC), Heterojunction with Intrinsic Thin Layer (HIT) technology, and bifacial solar cells have further improved the performance of solar panels. By optimizing the way solar cells capture and convert sunlight, these designs are helping to maximize energy output and make solar technology more viable in a wider range of environments, including urban settings.
In the area of manufacturing, advancements such as laser doping, kerfless wafer production, and the integration of automation and AI are making it possible to produce high-efficiency solar cells at scale. These innovations are crucial for reducing production costs and ensuring that solar energy remains competitive with other forms of energy.
Case studies from Germany and Austria have demonstrated the practical application of these technologies, showing how they can be successfully implemented in both large-scale solar farms and urban initiatives. These examples highlight the importance of site-specific solutions and the potential for solar energy to play a central role in meeting local and national energy needs.
Looking to the future, the integration of solar power with energy storage and smart grid technologies will be essential for overcoming the challenges of intermittency and ensuring a reliable energy supply. Additionally, policy and market dynamics will continue to shape the development of the solar industry, with government support and global competition driving innovation and adoption.
8.2 Importance of Continued Innovation
The ongoing innovation in solar technology is not just about improving efficiency or reducing costs; it is about fundamentally changing the way we produce and consume energy. As the world moves towards a more sustainable future, solar energy will play an increasingly important role in reducing greenhouse gas emissions, mitigating climate change, and providing clean, renewable energy to billions of people.
Continued investment in research and development is essential for maintaining the momentum of these advancements. This includes exploring new materials and technologies, refining manufacturing processes, and finding ways to integrate solar energy more effectively with other technologies and systems. Collaboration between research institutions, industry, and governments will be key to driving these innovations forward and ensuring that they are translated into real-world solutions.
8.3 Final Thoughts and Call to Action
As we look to the future, it is clear that the solar industry has the potential to become a dominant force in the global energy landscape. However, realizing this potential will require a concerted effort from all stakeholders—engineers, researchers, policymakers, and industry leaders alike.
For engineers and researchers, the challenge is to continue pushing the boundaries of what is possible, exploring new frontiers in material science, design, and manufacturing. For policymakers, the task is to create a supportive environment that encourages innovation while ensuring that the benefits of solar technology are accessible to all. For industry leaders, the focus should be on scaling up production, reducing costs, and integrating solar energy into the broader energy system.
Finally, for potential employers and industry professionals, there is a clear opportunity to engage with these cutting-edge technologies and contribute to the future of energy. Whether through research, development, or deployment, your expertise and commitment are vital to advancing solar energy and achieving a sustainable energy future.
The future of solar energy is bright, but it will require vision, dedication, and collaboration to fully realize its potential. Together, we can ensure that solar power becomes a cornerstone of the global energy system, providing clean, renewable energy for generations to come.